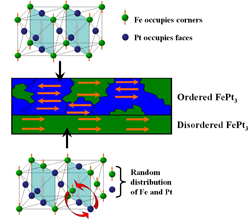
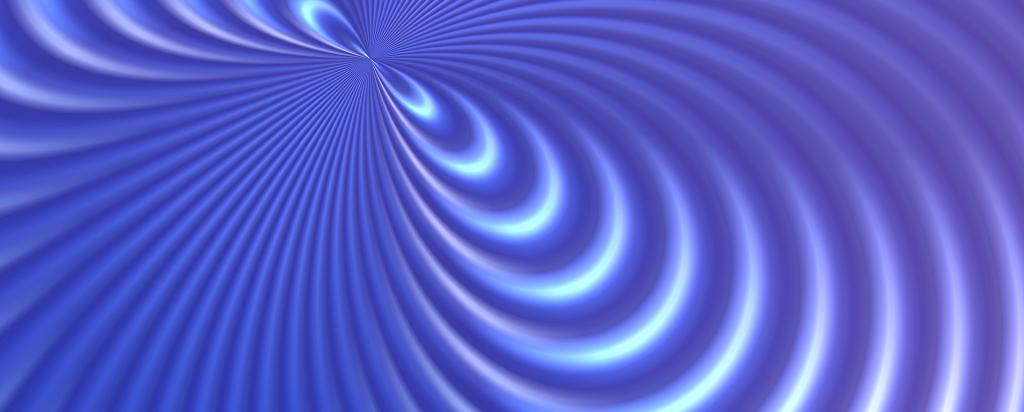
Magnetism
Although the neutron has no charge, it does have a magnetic moment, which can interact with the electronic magnetic moments of a crystalline material by diffraction to probe the magnetic structure, by inelastic scattering to probe the magnetic interactions, and even interact with the nuclear moments to add a further dimension to structural studies. Bulk magnetic properties are strongly linked to the interactions between the moments at the atomic level, and therefore neutron scattering can play a key role in developing better magnets for technological applications.
Principal Themes
Transition-metal Magnetism
Many transition-metal elements and compounds are strongly ferromagnetic at room temperature. Beginning with the archetypical magnetite or lodestone, transition metals have long formed the basis of most magnets for technological applications, thanks to the high crustal abundance of most transition-metal elements and their high variability of physical properties in stoichiometric combinations or by alloying. Colossal magnetoresistance, where the electrical resistance is changed dramatically in the presence of a magnetic field, is observed in some (mostly Mn-based) perovskite oxides.
Rare-earth Magnetism
Rare-earth elements are ferromagnetic but with Curie temperatures below room temperature. However they can form compounds with transition metals, and many of these compounds have Curie temperatures above room temperature. In addition the crystalline structures often have high magnetic anisotropy, which combined with the large atomic moments yields very strong permanent magnets. At present Nd2Fe14B is the strongest known permanent magnet. The current dwindling supply of rare-earth metals has intensified the search for alternate compounds and physical forms with smaller amounts of rare-earth metals, and neutrons have long played an essential role in guiding this search.
Actinide Magnetism
The unpaired electrons in the actinide series are the 5f electrons which often form into bands rather than exist as localised electrons. In addition there is a large inherent orbital moment which leads to strong spin-orbit interaction, comparable in magnitude to the bandwidth. Both properties lead to an extreme diversity of magnetic features in the actinide elements, and actinide-element-based alloys and compounds, ranging from itinerant magnetic compounds, through the exotic heavy-fermion materials, to fully localised compounds. Neutron scattering is the tool best suited to determine the degree of electronic hybridisation which is a key parameter in the characterisation of actinides.
While radioactivity and the associated health and security issues may limit the technological use of actinides and their compounds, the diversity of magnetic properties makes the series an excellent playground in which to test theories.
Magnetism in thin films and multilayers
Thin film structures, grown for example by sputtering deposition, chemical-vapour deposition or molecular-beam epitaxy (MBE), underpin the present-day electronics industry. It is also possible to produce magnetic multilayers that can be used in devices that exploit the spin rather than the charge of the electron. This new field of magneto-electronics or spintronics is revolutionising the electronics industry.
Already, spin-valve devices, based on multilayers that show giant magnetoresistance, are employed as read heads, and have led to dramatic improvements in data-storage densities. Semiconductor-based spintronic devices can combine storage, detection, logic and communication capabilities on a single chip to produce a multifunctional device that could replace several components in present devices. Diluted magnetic semiconductors (e.g. Ga1-xMnxAs) can be associated reasonably easily with non-magnetic semiconductors for electrical injection or detection of spin-polarised electrons.
At the level of basic science, thin-film growth may produce structures that are stable at lower temperatures than they are in the bulk, by use of an appropriate structural template as the substrate. Our understanding of the magnetism of matter is such that it is unlikely that new bulk alloys or compounds with significantly improved intrinsic properties may be discovered. Tailoring the magnetic properties at the nanoscale however offers much more perspective because the scale is comparable to the ferromagnetic interaction length. By alternating layers of metals with different magnetic properties, we can explore fundamental questions such as how magnetic order propagates across thin layers.
The magnetic coupling mechanism between the layers is often intricate, and can differ dramatically for small differences in the layer thicknesses. Artificial composite multiferroics can be constructed by multilayers of magnetic and ferroelectric materials, to produce, for example, tiny magnetic sensors. Neutron diffraction and specular neutron reflectivity are very well suited to investigate the coupling mechanism. Such studies are possible on epitaxial structures on the triple-axis spectrometer TAIPAN and on both epitaxial and polycrystalline thin-film structures on the reflectometer PLATYPUS.
For basic research, the structures are usually produced as repeated bilayers to produce a structure about 500-1000 Å thick. Structural characterisation of the repeated bilayer is conveniently made by three-axis X-ray diffraction. It is important to characterise the roughness of the interfaces, particularly since the preparation of multilayers has advanced to the point that multilayered structures with layers just two or three atomic planes thick may be produced.
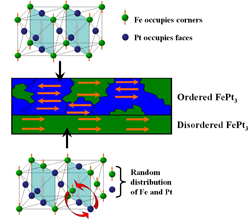
Chemically ordered/disordered bilayers of FePt3 show antiferromagnetic/ferromagnetic order
Molecular magnetism
Like epitaxy of metals, molecular engineering of organic compounds offers far more possibilities than traditional inorganic and metallic compounds to design magnets, and other smart materials, such as those with non-linear optical behaviour, with the desired physical properties for particular applications (density, maleability, transparency, solubility, photo-responsiveness, electrical conductivity, bio-compatibility, environment-friendliness, low-temperature fabrication, etc.). Magnetic molecules are also monodispersive, and can be diluted and organised using supramolecular techniques.
A major goal in molecular magnetism is to find molecular ferromagnets, but only a handful of purely organic ferromagnets have been found thus far, the first in 1991. As a consequence, investigation of molecular antiferromagnets is also important, in part to understand the laws governing the type of molecular magnetic ordering, including the role played by structural packing.
Single-crystal diffraction is usually preferred over powder diffraction for investigation of the crystallographic and magnetic structures of molecular materials because of the weakness of the magnetic signal, or the presence of hydrogen, which gives a large incoherent background.
Multiferroics
Multiferroics are materials which exhibit more than one ferroic order parameter (most commonly two of ferromagnetism, ferroelectricity, ferrelasticity), usually, but not necessarily, coupled, in a single phase. The coupling of the order parameters lends itself to novel device applications, where the form of the magnetic phase may be controlled by an applied electric field, or the electric polarisation controlled by an applied magnetic field.
In type-I multiferroics, ferroelectricity and magnetism have different sources, appear largely independently of one another, though there is some coupling between them, and ferroelectricity typically appears at higher temperatures than magnetism. In the more recently discovered type-II multiferroics, magnetism causes ferroelectricity, which implies a strong coupling between the two.
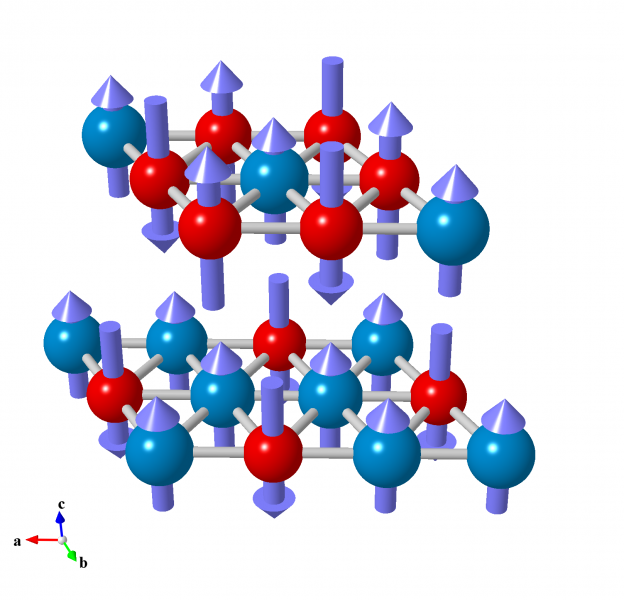
Antiferromagnetic ordering in LuFe2O4 stabilises ferroelectric charge ordering
Superconductivity
Magnetism and superconductivity are generally thought to be incompatible with each other. According to the Bardeen-Cooper-Schreiffer theory of superconductivity, electrons with opposite spins form pairs that can move through a material without resistance. A magnetic field can destroy superconductivity in two ways: by breaking up the electron pair, or by trying to make both of the electron spins point in the same direction.
These effects also limit how much current can flow through the superconductor because of the disruptive effect of the magnetic field produced by the current itself. Despite the known exceptions to this rule, a thorough characterisation of superconductivity is closely linked to characterisation of the magnetic behaviour. Studies of flux-line lattices in superconductors is a particularly active field in neutron scattering.