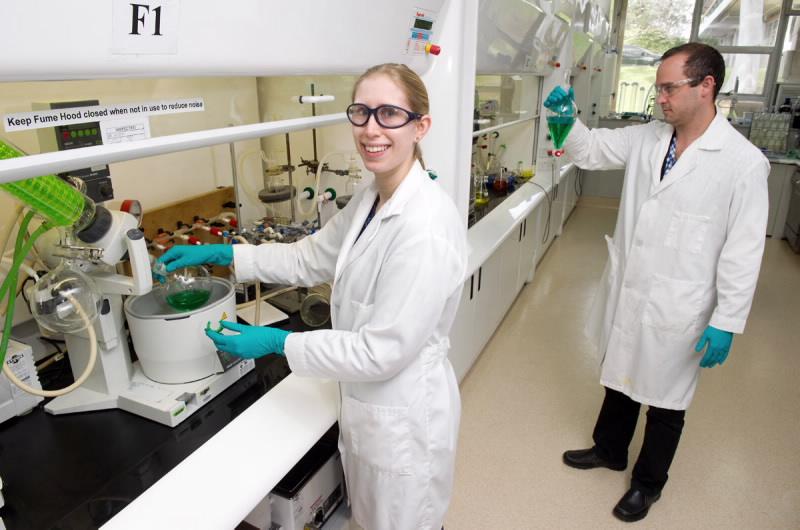
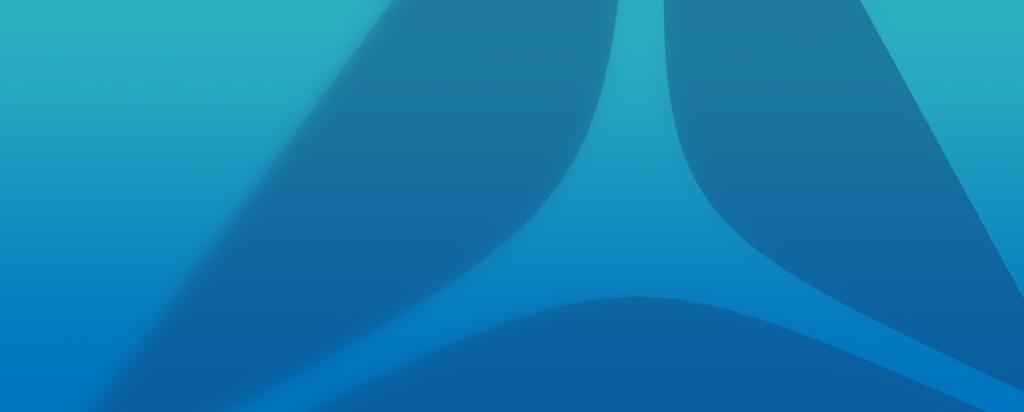
Published on the 12th June 2013 by ANSTO Staff
At ANSTO, radionuclides are central to nuclear medicine research and to answer fundamental questions in biology.
By Dr Benjamin Fraser
This year, the Australian Nuclear Science and Technology Organisation (ANSTO) celebrates 60 years since planning began to build Australia’s first nuclear reactor at Lucas Heights in southwest Sydney. Nuclear reactor technology has progressed significantly since the 1950s and the current Open Pool Australian Lightwater (OPAL) reactor is a state-of-the-art 20-megawatt nuclear reactor.
OPAL uses low-enriched uranium fuel (non-weapons grade), which demonstrates the Australian government’s ongoing commitment to worldwide nuclear non-proliferation. The neutrons produced by OPAL are used primarily for the production of radiopharmaceuticals, but also for neutron diffraction experiments and silicon doping.
Molybdenum-99 (99Mo) is the predominant radioisotope produced at OPAL and is the parent radioisotope to technetium-99m (99mTc), the world’s most commonly prescribed medical imaging radioisotope. 99mTc is used for diagnosing heart disease, cancer, and kidney and gastrointestinal tract disorders. Other applications include bone oncology and neurology investigation. In Australia, each year 550 000 people receive a diagnosis using 99mTc, and the current world demand is 45 million doses a year.
In September 2012, the Australian government announced a $168 million plan that will position ANSTO at the centre of the global fight against cancer and heart disease. The plan includes the development of a new export-scale nuclear medicine manufacturing plant, which will secure Australia’s ability to produce 99Mo and increase its capacity to meet a significant proportion of the world’s needs.
Although 99Mo production is probably the most important OPAL activity, the reactor also produces other exotic ‘neutron-rich’ radioisotopes for research purposes. When combined with the ‘proton-rich’ radioisotopes available from the National Imaging Facility (NIF) Camperdown cyclotron and other Australian cyclotrons, there is tremendous scope at ANSTO for undertaking new and exciting radiochemistry research.
Understanding the fundamental radiochemistry and developing applications of these new radioisotopes, including for incorporation into radiotracers and radiopharmaceuticals, is a key role of the radiochemistry group in ANSTO LifeSciences.
![]() |
Radiochemistry and synthetic chemistry
Radiochemistry is the study of the chemistry of radioactive atoms. For most purposes, different radioisotopes of a given element will have identical chemical properties and chemical reactivity. That is to say, a positronemitting radioisotope such as fluorine-18, will behave identically, in a chemical sense, to non-radioactive fluorine-19. There are some rare exceptions to this rule such as the significant difference in reaction rates observed between molecules that contain either deuterium or hydrogen substituents (the kinetic isotope effect).
Some of the most important differences between radiochemistry and conventional synthetic chemistry relate to issues of stoichiometry, concentration, reaction times and purification techniques.
Radiochemistry is typically performed with very small amounts of radioactive atoms in very dilute concentrations (nanomolar to picomolar). Any reagent reacting with the radioactive atoms is typically in vast excess (tens of thousands of equivalents), which leads to pseudo first-order kinetics and fast reactions. This is essential because radioisotopes such as carbon-11 (t1/2 = 20 minutes) and gallium-68 (t1/2 = 68 minutes) have relatively short half-lives (the time taken for half of the radioactive atoms in a sample to decay). Removal of the resulting impurities, particularly for small molecule radiotracers, is often performed by high-performance liquid chromatography (HPLC).
Conventional purification techniques such as crystallisation and trituration are not practical and virtually impossible. Finally, contamination of the ‘hot’ radioisotope with ‘cold’ isotope must be minimised. Although this will not affect the final radiochemical yield, the radiotracer may be rendered ineffective.
Although radiochemistry is our main focus, the radiochemistry group at ANSTO still does plenty of conventional synthetic chemistry. Chemical precursors for radiolabelling experiments are prepared in ‘normal’ synthetic laboratories.
Radiopharmaceuticals and emission tomography
Radiopharmaceuticals are a subset of radiotracers (compounds labelled with radioactive atoms) that are used to diagnose or treat disease. They are made up of two components – a radioisotope (e.g. fluorine-18, technetium-99m) attached to a chemical entity (such as small molecule, peptide, protein or antibody). The chemical entity facilitates transport and uptake of the radioisotope in the body. Radiopharmaceuticals are typically administered in very low doses (unlike normal pharmaceuticals) and often have no pharmacological effect, including no side effects.
There are two general classes of radiopharmaceutical – imaging and therapeutic. Imaging radio - pharmaceuticals are composed of a radionuclide that emits radiation (most often gamma radiation) that is detected and used to generate a medical image. We develop new radiotracer tools and radio - pharmaceuticals based on two nuclear imaging modalities: single-photon emission computed tomography (SPECT) and positron emission tomography (PET). It would take too long to explain all nuclear imaging modalities, so we’ll focus on the most recent technology.
PET is a powerful nuclear imaging technique that gives detailed three dimensional images of functional processes in the body. A PET radiopharmaceutical is administered to a patient and then given time (depending on the type of scan) to distribute through the body, accumulate at sites of interest. The patient is then placed in a PET scanner and a series of images is generated.
At the molecular level, at any moment in time, one of the PET radionuclides can decay and emit a positron. That positron travels a certain distance (~1 mm) and then slows down and annihilates by colliding with an electron. This event produces two gamma rays that are separated by 180°. The simultaneous detection of millions of these gamma ray pairs allows precise determination of the distribution of the radiopharmaceutical in the body.
Radioisotope | Half life | Decay mode | Source | Uses |
Carbon -11 | 20min | >99% positron emission <1% electron capture | Camperdown cyclotron ^ 14N(p,α) 11C | Pet imaging Neuroimaginh |
Gallium-68 | 68min | 89% positron emission | 68Ga generatorB (68Ge parent) | Pet imaging Tumour imaging |
Fluorine- 18 | 109min | 97% positron emission 3% electron capture | Camperdown cyclotron^ 18O(p,n) → 18F | Pet imaging |
Technetium-99m | 6 h | >99% isomeric transition | 99mTc generatorC 99Mo parent | SPECT imaging |
Zirconium-89 | 78 h | 25% positron emission 75% electron capture | Australian cyclotronD 89Y(p,n) → 89Zr | PET and immuno PET imaging |
Lutetium-177 | 6.7 days | 81% beta emission 19% gamma emission | OPAL reactorC 76Yb(n,γ) → 177Yb → 177Lu | Therapy immunotherapy |
Iodine-125 | 60 days | 100% electron capture | ReactorB 124Xe(n,γ) → 125mXe →125I | Biological assay Brachytherapy Thyroid imaging |
When, where and how fast the radiopharmaceutical accumulates in the body (due to metabolic uptake, clearance, receptor/enzyme binding etc.) gives detailed physiological and biochemical information. This information is then used to diagnose human disease. We also perform preclinical radiopharmaceutical development on small animal PET/CT scanners, which combine the detailed physiological information from a PET scan with the anatomical detail from a computed tomography (CT) scan.
Therapeutic radiopharmaceuticals are composed of a radionuclide that emits radiation (most often beta or alpha radiation) that destroys cells, which provides a therapeutic benefit to the patient. Lutetium-177 is a relatively low-energy beta emitter that has been attached, via a DOTA-based chelating ligand, to octreotide (a peptide mimetic). This selectively binds to receptors that are over-expressed by endocrine tumours. In this way the lutetium-177 accumulates and delivers significantly more radiation dose to the tumour than the surrounding tissue. This differential dose will hopefully kill the tumour cells but leave ordinary cells intact.
We are preparing new types of chelating ligands for lutetium-177. Our goal is to prepare new ligands, and ultimately develop radio- pharmaceuticals, that have better selectivity and affinity for lutetium than current ligand systems. This should increase the therapeutic benefit by ensuring the tumour receives the maximum radiation dose while minimising the background dose to the patient.
![]() |
Principles of positron emission tomography (PET) imaging |
Half-life and the ‘perfect’ radioisotope
Half-life (t1/2) dictates what type (and how many) chemical reactions, manipulations and/or purifications can be performed on a sample. We work with radioisotopes with large variations in half-life. Carbon-11 is one of the shortest (20 minutes), zirconium-89 is intermediate (78 hours) and iodine-125 is one of the longest (60 days) (see table). Fluorine-18 is often described as the ‘perfect’ radioisotope for PET imaging because of its low-energy positron emission (0.202 MeV), small size (C–F 1.35 Å, C–O 1.43 Å), excellent decay profile (97% positron emission) and intermediate half-life (109 minutes). This means there is enough time to transport the radioisotope and perform multi-step syntheses; however, it is not long enough to incorporate it into radiopharmaceuticals where the chemical entity (e.g. antibody) can take days to circulate and accumulate at the target (e.g. tumour).
ANSTO LifeSciences is developing new ligand systems that have high affinity and selectivity for PET radioisotopes with longer half-lives such as zirconium-89 (78 hours) and scandium-44 (4 hours). Copper-64 is also a PET radioisotope with a longer half-life (12.7 hours) and significant potential for medical imaging. Copper- 64, however, has several other decay modes, which could lead to an unacceptably high radiation burden on the patient. Although gallium-68 has a shorter half-life (68 minutes) and is not suitable for this application, we are very interested in this radioisotope as it is available from a generator. The parent isotope, germanium-68, is cyclotron produced and has a long half-life (271 days), making the generators useful for 1–2 years.
Radiochemical yield and microfluidic reactors
Radiochemical yield is the amount of radioactivity in the final compound expressed as a percentage of the starting radioactivity. Radiochemical yields close to 100% are possible as they are often expressed in decaycorrected terms (which take into account loses due to decay). Nondecay- corrected radiochemical yields are often less ‘impressive’, but are more useful because they more accurately reflect the true yield of the radiolabelling reaction. Radiochemical yield can generally be increased in two ways: by increasing the efficiency of incorporation of the radionuclide and by shortening the reaction time.
We are pioneering the use of microfluidic reaction technology along with a small number of other international research groups.
Microfluidic reactors have internal diameters of 10–500 mm and have much larger surface areas than conventional vessel-type reactors. As a result, microfluidic reactors mix fluids with high speed, facilitate rapid heat transfer, give shorter reaction times, reduce consumption of reagents and reduce exposure to ionising radiation because of the ease of shielding.
Given these benefits, we envisage microfluidic reactor technology will become a standard tool for radiochemistry research in the future. Specific activity and scale-up Specific activity is the amount of radioactivity in a sample per amount of substance. It is usually expressed as becquerels per kilogram (1 becquerel is one disintegration per second). High specific activity is very important and is achieved by minimising contamination of the ‘hot’ radioisotope with ‘cold’ isotope. For example, a [18F]fluorine-based radiopharmaceutical would never be prepared using tap water because tap water usually contains vast amounts (on a radiochemical scale) of ‘cold’ [19F]fluoride. Contamination with large amounts of ‘cold’ isotope leads to low specific activity, which generally renders the [18F]radiopharmaceutical useless as an imaging agent, especially when the target (e.g. receptor, enzyme) expression is low.
![]() |
Therapeutic radiopharmaceutical [177Lu] - DOTATATE |
In ANSTO LifeSciences, we develop automated methods for large-scale preparations of radiotracers and radio -pharmaceuticals. Higher specific activities are generally achieved with larger amounts of radioactivity, but this also increases the radiation dose to the operator. Automated modules, which are operated remotely by the radiochemist, who is completely shielded, reduce this risk. We endeavour to develop radiolabelling methodologies on various commercially available automation modules so that our published procedures can be easily reproduced for future animal studies and clinical trials.